Audio amplifier evaluation parameters
Premise
In electronics, an amplifier is a device that increases signal strength. Specifically, voltage amplifiers increase the amplitude of an input signal, while current amplifiers increase the available current while maintaining the same amplitude. Audio amplifiers fall into the voltage amplifier category, ideally functioning as constant voltage sources.
Unlike general-purpose amplifiers, audio amplifiers have specialized requirements. They must accurately process frequencies within the human hearing range (typically 20Hz to 20kHz), drive complex loads such as loudspeakers, and handle signals that constantly vary in both amplitude and frequency. This complexity means that properly characterizing an audio amplifier’s performance requires examining multiple parameters.
Unfortunately, the audio industry has historically overemphasized a single measurement: Total Harmonic Distortion (THD). This focus has created a division in the audio community. One group places complete faith in amplifiers with extremely low measured distortion values, while another group has grown skeptical of measurements altogether, noting that amplifiers with similar distortion specifications can sound noticeably different when handling real-world signals and speaker loads.
This disconnect has led some audio enthusiasts to abandon scientific measurement entirely, sometimes embracing pseudoscientific concepts like exotic cables or unusual grounding methods. The root issue is inadequate education about amplifier performance metrics and their practical significance.
Encouragingly, there is a gradual shift toward more comprehensive scientific evaluation of audio equipment. However, progress remains slow. The key takeaway is that evaluating an amplifier’s performance requires considering multiple parameters—not just distortion—to predict how it will behave with different input signals and output loads.
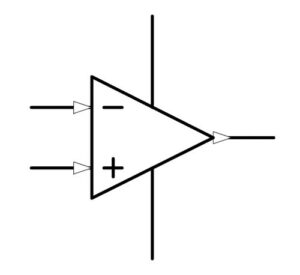
Amplifier classes
Unlike household appliance ratings where classes directly indicate energy efficiency, amplifier classes describe how the device operates, with efficiency being a consequence of that operation method.
An audio amplifier’s class defines two fundamental characteristics:
- The method of operation (linear amplification or switching)
- The percentage of input signal being amplified (ranging from 0% to 100%)
It’s important to understand that class designations can apply at different levels – to individual components (such as tubes, MOSFETs, or transistors), to specific amplification stages, or to the entire amplifier system.
Crucially, an amplifier’s class is not an indicator of sound quality. Rather, the class determines specific operational characteristics and limitations, particularly regarding efficiency and response speed. Different applications may benefit from different amplifier classes depending on their specific requirements.
Class operation methods
Linear amplification
A linear amplifier uses simple devices, as transistors, tubes or mosfets to directly increase the input signal.
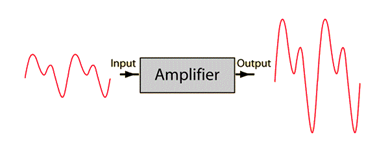
The input signal directly commands the amplification device, that increase the current flow at the output. In a transistor, this is done by adding or subtracting (depends on polarization) current at its base: this cause the electrons flow to increase through the device.
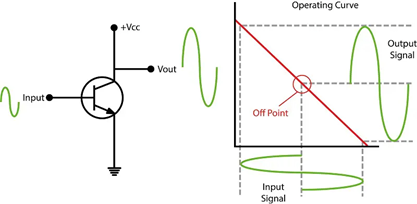
Switching amplification
Switching amplification is a more complex amplification.
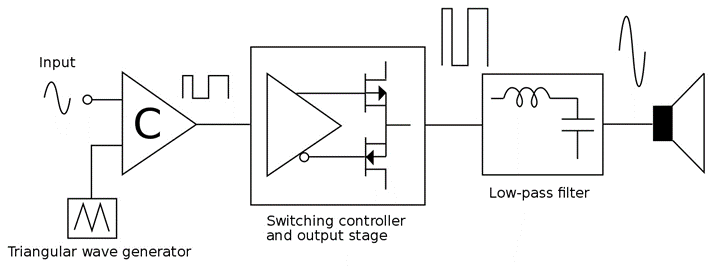
The input signal is summed with an high frequency triangular wave through a comparator, obtaining a square wave with variable duty cycle in function of the input signal.
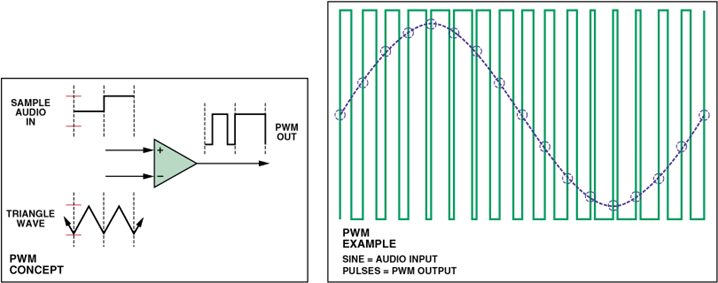
Then the square wave is linearly amplified with high frequency devices as MOSFETs, and lastly the signal is filtered by a low-pass LC (inductor-capacitor) filter to return the original signal.
Classes
Class A
Class A is the first amplifier class created. The device, or the amplifier stage is always fully-opened: the signal is always within the current flowing through the device or stage.
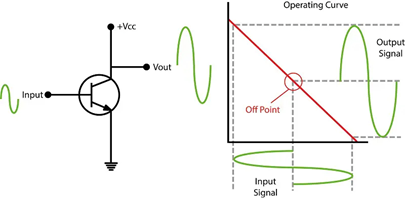
Due to class amplification method, the efficiency is really low.
- Operation method: LINEAR
- Maximum efficiency: 25%
- Signal conduction: 100%
Class B
Class B conduct only half of the signal, it was used to greatly reduce the heat generated by class A operation, but it requires minimum two devices to achieve the full conduction of signal.
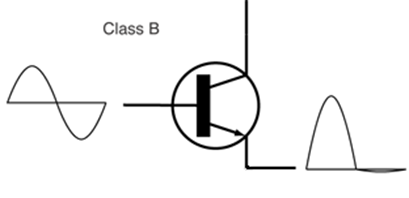
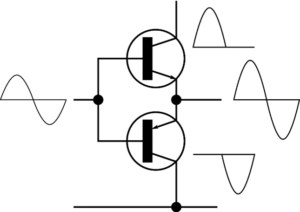
This permit to open the device only when the signal is put in the input, and so greatly increase the efficiency.
- Operation method: LINEAR
- Maximum efficiency: 78.5%
- Signal conduction: 50%
Class AB
In theory, class B is working fine: when the signal is positive then the upper device is working and when is negative then the upper stop working and the lower device start working.
There is a problem in practice: below a certain voltage threshold, the devices do not conduct. So when the signal approaches the crossing point between the positive and negative parts, there is a period of time where the signal is not conducted. Resulting in the typical distortion called “crossover distortion”.
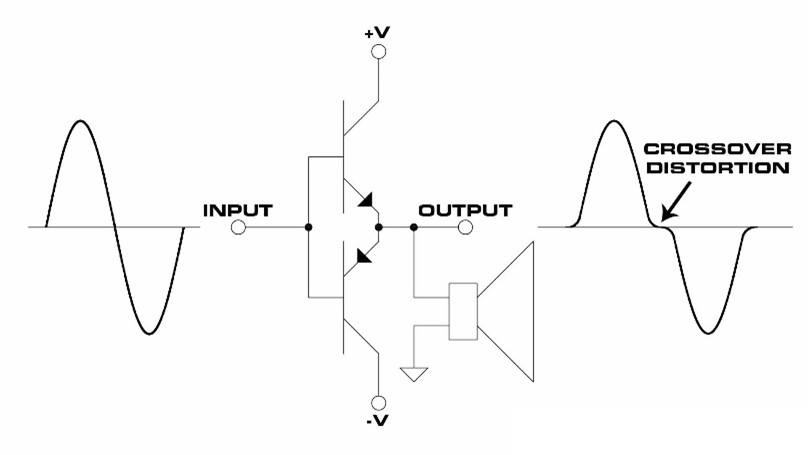
Consequently, class AB tries to sum class A with B: the devices are kept partially switched on above their minimum non-conduction threshold, below this threshold the signal is conducted entirely (in class A), above this threshold it is conducted partially (in class B).
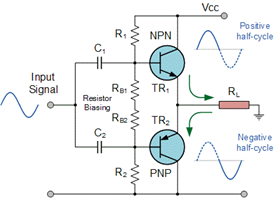
This greatly reduces crossover distortion, but does not completely eliminate it.
- Operation method: LINEAR
- Maximum efficiency: 78.5%
- Signal conduction: >50% U <100%
Class G & H
Class G and class H were created to maintain the operation method linear and maintaining the same performances, but also to increase efficiency.
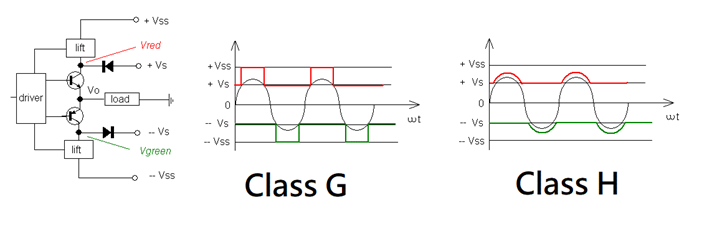
The concept is that the devices in class B/AB act as power regulators: the internal area of the signal function is power in output, and everything outer till the supply voltage is dissipated power.
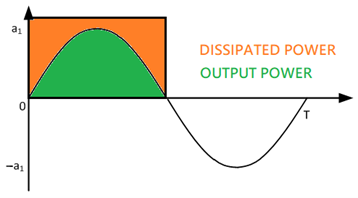
Class G and class H use more power supply rails, to rise the voltage when needed, so the amplifier can achieve theoretically the maximum efficiency.
- Operation method: LINEAR
- Maximum efficiency: 85.9% (if class G) | >85.9% U <=100% (if class H)
- Signal conduction: >50% U <100%
Class D & T
Both class D and T work with the switching mode operation. The reason for using such a complex amplification method is its efficiency. Class D were originally created for pro and PA audio, so a lot of power was needed in tight spaces and with little heat dissipation.
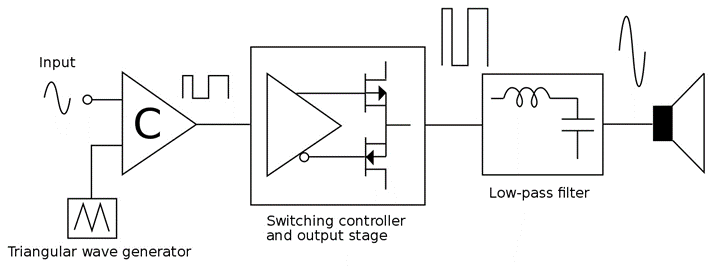
Originally this class had far inferior performances to the linear classes, the biggest problem was a distortion created by the on and off misalignment between the upper and lower devices. This distortion is referred to as “dead time”.
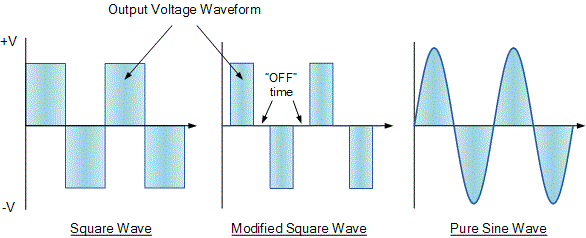
Class T amplifiers were developed as an improvement to traditional switching amplifiers, specifically addressing two critical limitations: reducing dead time (non-conductive periods) and enhancing synchronization precision. Over time, the separate “Class T” designation faded, with all such designs now falling under the broader Class D category. Modern Class D amplifiers have evolved dramatically, now delivering performance that exceeds many medium-quality linear amplifiers.
- Operation method: Switching
- Maximum theoretical efficiency: 100%
- Signal conduction: 100%
While amplifier classes themselves don’t determine sound quality, switching amplifiers face unique engineering challenges. Contrary to what might be expected, these challenges aren’t primarily related to traditional audio specifications like distortion levels, signal-to-noise ratio, or output impedance. Rather, the fundamental limitation involves speed—specifically how quickly the amplifier can respond to rapid signal changes.
This speed limitation is better understood through the “Slew rate” parameter, which will be explained in more detail below.
Amplifier parameters
Amplifier performance parameters can be divided into two distinct categories:
Acoustic Parameters
These parameters describe how an amplifier processes musical signals and manages its load (speakers), directly affecting what we hear. Acoustic parameters help us understand the actual listening experience and sound quality.
Non-Acoustic Parameters
These parameters relate to implementation and practical considerations, such as:
- Power supply compatibility
- Energy efficiency
- Thermal stability
- Physical design considerations
An Important Clarification
There’s a common misconception that certain parameters apply differently to different amplifier classes. This is incorrect. Regardless of the amplifier class (A, AB, D, etc.), the same parameters remain valid and relevant.
What truly matters is not how an amplifier achieves certain performance metrics internally, but rather:
- What the connected speakers (load) actually receive
- How effectively the amplifier manages the complex electrical characteristics of those speakers
The fundamental principles of audio reproduction apply universally across all amplifier technologies.
[1] Acoustic | Distortion of a fundamental signal (THD)
Distortion measures how much an amplifier alters or “dirties” the original signal during the amplification process. Simply put, it quantifies the unwanted changes introduced to the audio.
How Distortion Is Measured
Standard measurement involves inputting a pure sinusoidal tone at 1kHz with constant amplitude. The test setup varies by amplifier type:
- For power amplifiers: An 8 Ohm non-inductive resistive load simulates a speaker
- For headphone amplifiers: Similar resistive loads between 32-600 Ohms are used
Understanding Distortion Values
Distortion is expressed as a percentage representing the ratio between:
- The voltage of the original (fundamental) signal
- The voltage of unwanted harmonics generated during amplification
These harmonics are byproducts of the amplification process itself. The larger these harmonics are relative to the fundamental signal, the higher the distortion percentage, indicating less accurate signal reproduction.
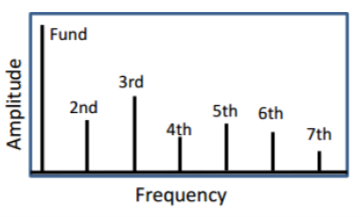
Distortion typically increases in predictable ways:
- In linear amplifiers, it rises approximately 6dB per octave as frequency increases
- In all amplifiers, it increases with both signal amplitude and frequency
- It worsens as speaker impedance decreases
While a single distortion measurement provides limited insight, analyzing distortion patterns can reveal:
- How an amplifier handles challenging low-impedance loads
- Potential crossover distortion issues
- Whether an amplifier is underbiased, correctly biased, or overbiased
Some amplifier modifications can be counterproductive. For instance, simply increasing the quiescent current to convert a Class AB amplifier to Class A operation often leads to problems. True Class A amplifiers must be designed as such from the outset. Excessive bias current (overbiasing) can actually increase even-harmonic distortion.
When distortion becomes audible, its character depends on which harmonics predominate:
- Even harmonics (2nd, 4th, etc.) are in phase with the fundamental and create a “sweeter” sound
- Odd harmonics (3rd, 5th, etc.) are out of phase and produce “shrill,” potentially fatiguing sound
These effects are most noticeable when the harmonics fall within the audible range and are relatively close to the fundamental frequency. Regardless of type, higher levels of harmonic content generally indicate poorer reproduction quality.
Distortion fundamentally represents degradation of the original signal and remains one of the primary indicators of an amplifier’s performance quality.
[2] Acoustic | Intermodulation distortion (IMD)
Intermodulation distortion is measured in the same way as fundamental distortion: it is the result of the ratio between the introduced signal and the harmonics produced. However, in its measurement, two or more signals are introduced simultaneously with the same amplitude but different frequency.
Some signal generators and spectrum analyzers introduce over 10-15 signals simultaneously. The more signals are introduced simultaneously, the more precise the measurement of the intermodulation distortion.
It is important to note here that for the IMD distortion measurement to be effective, the fundamental signals’ frequencies must not be the same as the possible harmonic frequencies of the other signals. For example, the possible harmonics for 1kHz are 2kHz, 3kHz, 4kHz etc. If the second signal has a frequency of 3kHz, then it will hide the harmonic amplitude in that position and will offset the measurement (with the second signal’s harmonics too).
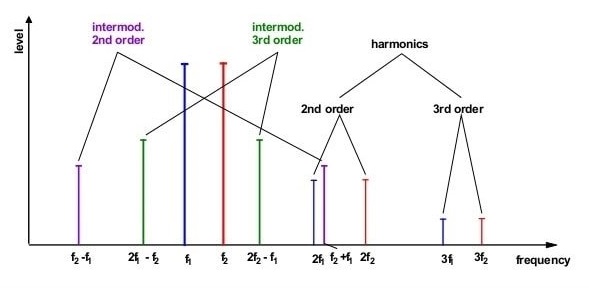
The intermodulation distortion has a high impact on the performance of the amplifier, and is a value indicative of its accuracy.
The validity of this measurement is greater than the simple fundamental, because it makes us understand how the amplifier behaves with a signal closer to musical content. However, all fundamentals are still constant in magnitude, which differs from actual music signals that continuously vary.
[3] Acoustic | Thermal and memory distortion
Thermal and memory distortions have nothing to do with the previous ones, despite being measured with a similar method.
To explain how it works, it must be understood that an electronic component varies its performance and parameters as the temperature changes or as signals pass through it (causing current flow). For example, a resistor may deviate from its nominal value, or a transistor might experience changes in gain or internal resistance.
This type of distortion indicates how well an amplifier maintains consistent performance despite temperature fluctuations in components or variations in the input signal. The smaller these performance variations, the lower the thermal and memory distortion.
A component’s temperature changes as signal levels vary, and importantly, any thermal errors get multiplied by the component’s gain. This is why it’s particularly critical to address thermal errors in stages or components where gain is very high.
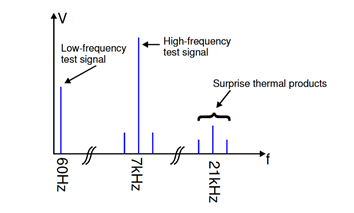
Normally, thermal distortion is simulated through temperature sweeps between components. Otherwise, one of the methods to measure it in reality would be to introduce two or more non-regular amplitude signals to the input and verify their output over time.
This parameter strongly determines the enjoyment of the outgoing musical signal. This is the strong point of tube amplifiers, as the gain of the stages is usually very low and the temperature at the passage of the signal is almost constant. However, if a solid-state amplifier is designed with sufficient attention to thermal management, the thermal distortion can certainly be much lower than that of tube amplifiers.
[4] Acoustic | Hysteresis distortion
Hysteresis distortion is a type of distortion typical of inductors with magnetic core, for example, it affects the 99.9% of switching amplifiers (class D, T) in commerce and all the linear amplifier who have an output inductor with cores.
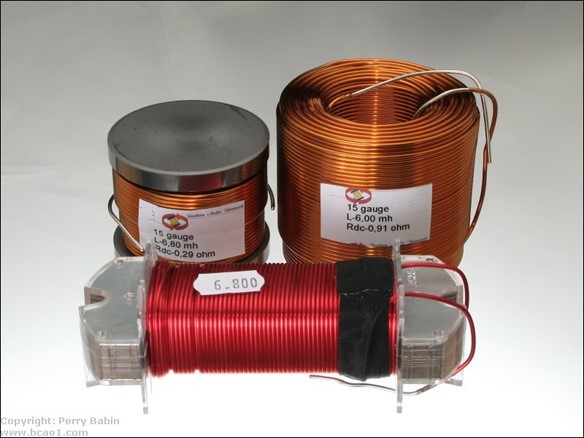
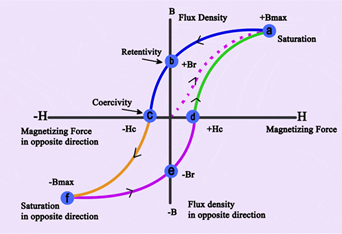
It has always been known in the world of electronics and signals, and lately it is much discussed in the world of class D amplification.
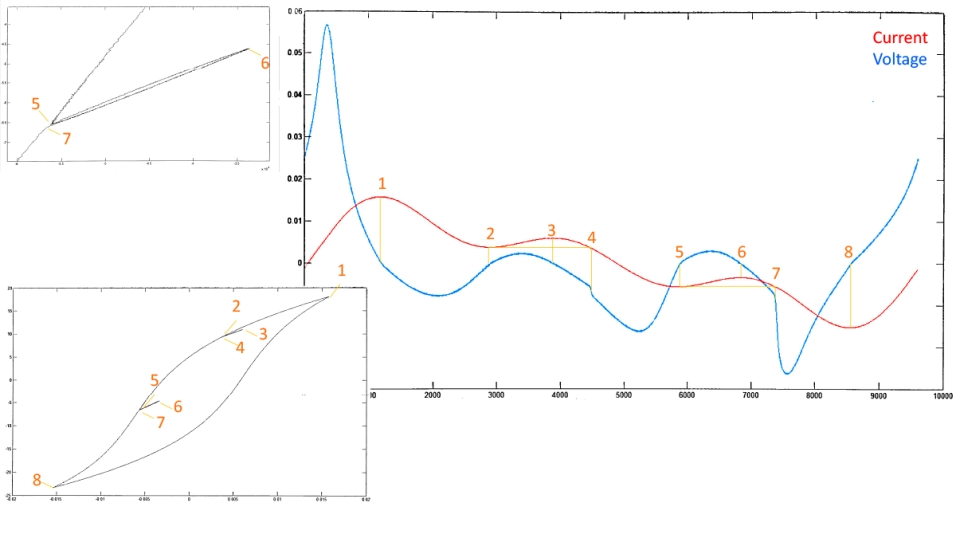
The figure above highlights the issue. The signal traverses the B-H curve in each direction as given in the graph. The flux (and thus the voltage) at a specific point on the curve can be different depending how you arrive at that point. For instance, following the path 5 to 7 takes you through point 6, and although points 5 and 7 are identical on the B-H curve, the voltage at that point is not. The same situation occurs when you go from 2 to 4 through 3; the voltage values at identical points 2 and 4 are different depending on the path you took to arrive there. Clearly, there are voltage ‘jumps’ when, after traversing a minor B-H loop, you arrive at the same point at the major loop where you were before. So, there is an element of which causes strong non-linearity.
[5] Acoustic | Damping factor (DF)
The effects of damping factor on the loudspeaker are better described in the article Damping Factor effects on loudspeakers. We invite you to read the full article about the damping factor effects and how it works to better understand.
[6] Acoustic | Slew rate (SR)
The effects of slew rate are better described in the article Slew rate effects on audio. We invite you to read the full article about the slew rate effects and how it works to better understand.
[7] Acoustic | Bandwidth and phase
The bandwidth is nothing more than the frequency response of an electronic device. In the case of an audio amplifier, it is measured by observing the output voltage obtained with a signal of constant amplitude at the input but varying frequency.
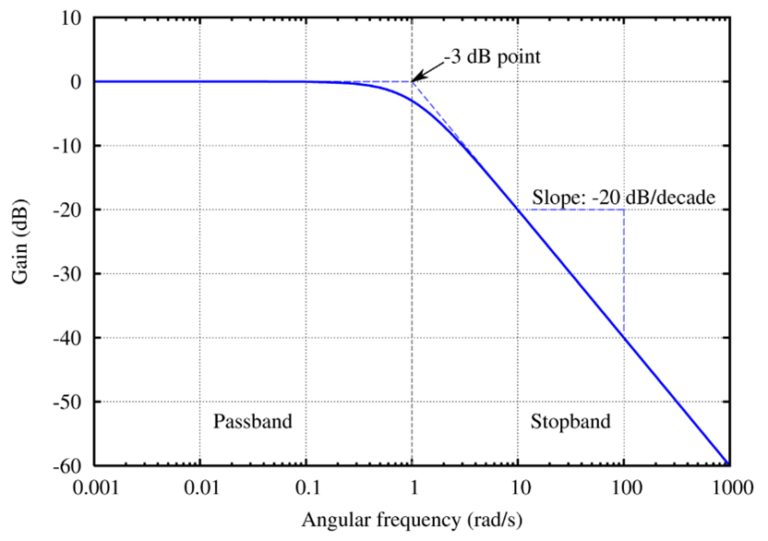
The band is considered the set of frequencies reproducible by the amplifier with consistent output level. The band extremes are defined when the output voltage (always with the same input voltage) drops to half of its nominal value, known as the -3dB point.
The nominal voltage obtained with the same input is called “gain” and is expressed in decibels. If an amplifier has 27dB of gain, it means that it amplifies the input voltage by approximately 22 times within its specified bandwidth.
The reason the output voltage decreases as frequency increases is that an amplifier does not have infinite speed. In fact, bandwidth and slew rate are extremely linked to each other: to reproduce a higher frequency at the same voltage, greater speed is necessary, otherwise the output voltage decreases. For this reason, low-bandwidth amplifiers typically also have limited slew rates.
As the frequency increases while speed remains constant, the voltage decreases. This means that current is delivered to the load with a delay compared to when it should arrive: the amplifier becomes increasingly unable to meet the current requirements of the load as frequency increases. This creates an effect defined as “phase shift.”
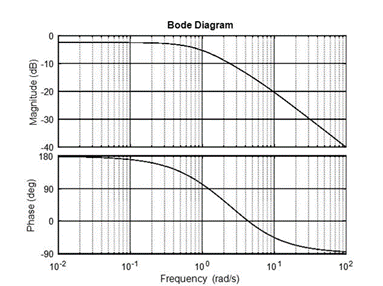
The phase shift of a frequency is a completely normal behavior and is part of every frequency response, regardless of the implementation of the components and independent of the electronic device. In the case of an audio amplifier, a phase change corresponds to a shift of the transient over time.
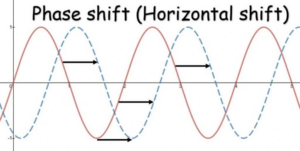
The greater the phase shift, the greater the displacement of the transient in time: in practice, the transient experiences a delay in its reproduction compared to its original position.
This can become a significant problem in amplifiers with very narrow frequency bands: the narrower the band, the greater the phase shift within the audible frequency range. Very wide frequency bands are often considered unnecessary in audio since human hearing only extends to about 20kHz. However, extended frequency response allows for minimal phase shifts and supports higher slew rates.
In some cases, narrow frequency bands can also cause attenuation within the audible range if the roll-off slope is too gradual and the cutoff point is positioned too close to the audible band.
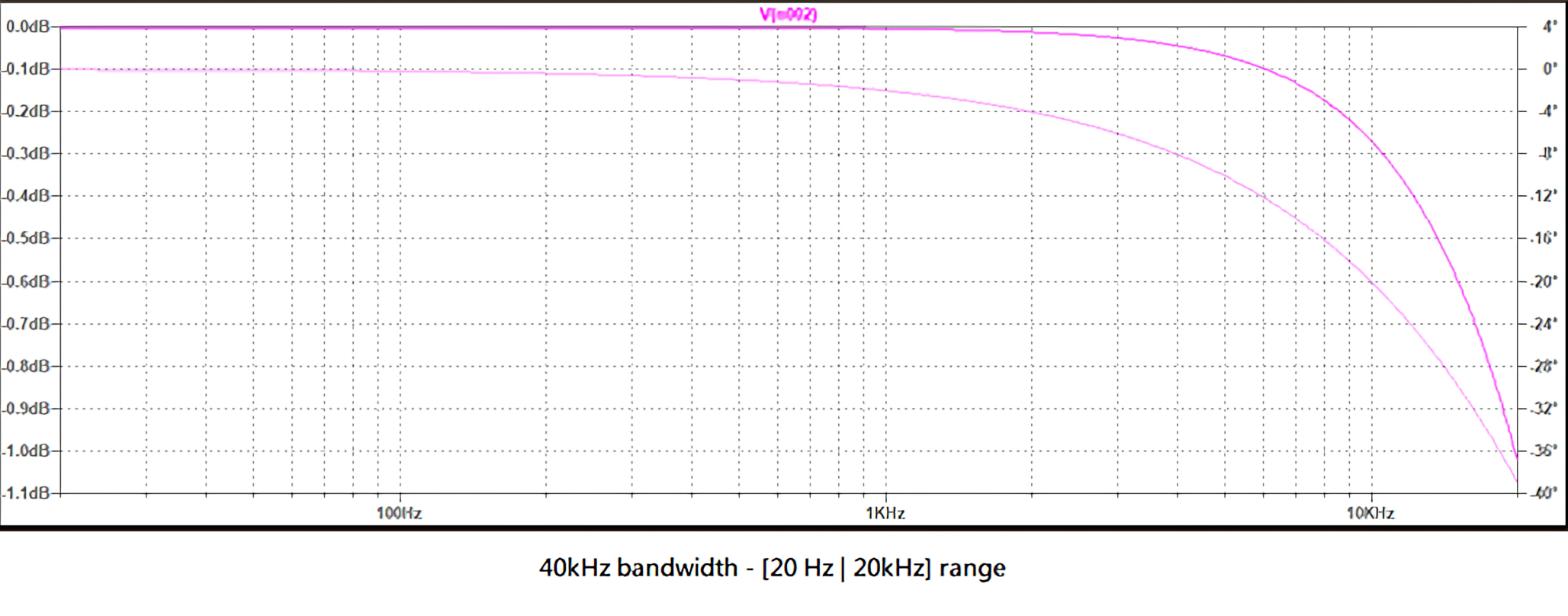
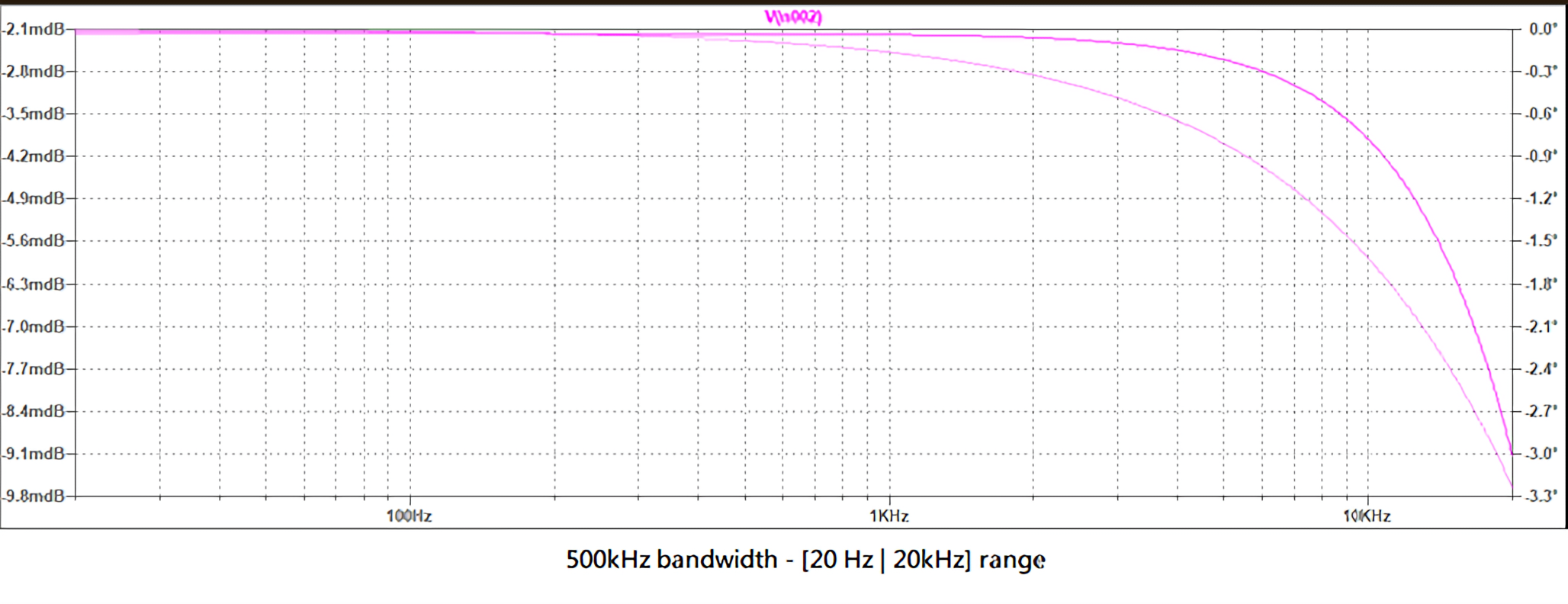
With a band of only 40kHz there is a phase shift of 40° at 20kHz, with a band of 500kHz there is a shift of only 3° and almost zero attenuation.
The absolute phase would be of little importance if the amplifier were to reproduce a single frequency. However, an audio amplifier must reproduce several frequencies together (musical signal), and if the phase causes a transient to shift significantly, it ends up in the wrong time position relative to other transients. This results in staggered playback where different frequency components of the same sound arrive at slightly different times, compromising the accurate reproduction of the original musical event.
[8] Acoustic | Power bandwidth
While standard bandwidth measurements are typically taken at low input voltages, power bandwidth is a much more critical specification for real-world performance. Power bandwidth depends directly on both the amplifier’s slew rate and the connected speaker load. Unlike standard bandwidth, power bandwidth changes as output voltage increases.
An important relationship exists: as output voltage increases, power bandwidth decreases. This specification tells you the maximum frequency an amplifier can reproduce at a specific output voltage level with its given slew rate.
This distinction matters because an amplifier might boast an impressively wide frequency response in its specifications, but if it has a limited slew rate, it won’t be able to deliver its full rated power across the entire audible frequency range. In practical terms, this means bass frequencies might receive full power while higher frequencies experience power compression.
[9] Acoustic | Signal to Noise ratio (SNR)
The Noise Floor refers to the background noise an amplifier produces when no input signal is present or when its input is connected to ground. This is what you hear from your speakers when the amplifier is powered on but not playing any music—the inherent electronic “hiss” of the system.
Signal-to-Noise Ratio (SNR) measures the relationship between your desired audio signal and unwanted noise generated during amplification. This noise can either increase or sometimes decrease as input signal levels change. SNR is expressed as the voltage difference between the fundamental signal and the generated noise, and should not be confused with harmonic distortion. A higher SNR indicates a “quieter” amplifier with less background noise.
Interestingly, an amplifier’s SNR doesn’t necessarily correlate with its distortion characteristics. It’s quite common to find amplifiers with excellent (high) SNR measurements that actually produce more distortion than models with somewhat lower SNR values. This highlights why multiple parameters must be considered when evaluating amplifier performance.
[10] Acoustic | Power factor
The Power Factor represents an amplifier’s actual output power at a specific frequency. This parameter is determined by the phase relationship between output voltage and current. When voltage and current are perfectly aligned (0° phase difference), the Power Factor equals 1, indicating maximum power transfer efficiency.
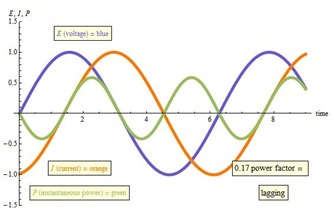
Importantly, the Power Factor isn’t solely determined by the amplifier itself—it’s significantly affected by what’s connected to it. Inductive elements and passive crossover capacitors in speakers will always reduce the Power Factor from its ideal value.
This parameter substantially influences how we perceive an amplifier’s bandwidth sensitivity. The Power Factor can be diminished by several deficiencies: insufficient slew rate, limited bandwidth, inadequate damping factor, and consequently poor control over the connected loudspeaker. When any of these parameters are suboptimal, the amplifier’s ability to deliver its maximum potential power is compromised.
[11] Non-acoustic | Input impedance
The input impedance of an amplifier represents its resistance to incoming signals. Theoretically, since an amplifier functions as a voltage generator, this value should be infinite. In real-world applications, however, practical limitations exist.
While input impedance is sometimes discussed as if it directly affects sound quality, it actually only becomes audibly noticeable when it’s inappropriate for a given setup. When input impedance is too low, the source device may struggle to deliver sufficient current to properly drive the amplifier, resulting in signal degradation. Conversely, excessively high input impedance makes the amplifier more susceptible to external interference and noise.
For these reasons, most well-designed audio amplifiers feature input impedance values between 10kOhm and 47kOhm. This range represents an ideal compromise that works effectively with virtually any audio source while maintaining minimal input noise.
[12] Non-acoustic | Output maximum voltage
The maximum output voltage of an amplifier is directly related to its power supply voltage. Put simply, the higher the voltage used to power the amplifier, the higher the potential output voltage it can deliver to speakers or headphones. This relationship exists unless the amplifier includes internal circuitry that deliberately limits the maximum output, which some designs incorporate for protection or other technical reasons.
[13] Non-acoustic | Output maximum current
The maximum output current an amplifier can deliver depends on two primary factors: the Safe Operating Area (SOA) of its output devices and its power supply voltage. The SOA defines the operational limits within which output components can function safely without damage.
Generally, having more output devices connected in parallel increases available current. However, there’s also an inverse relationship with supply voltage—at lower supply voltages, an amplifier can typically deliver higher current while still operating within the safe limits of its components.
It’s important to understand that current capability varies significantly between different device models. A single pair of high-current output devices might actually deliver more current than multiple pairs of a different, lower-capacity model. This is why the specific components used matter as much as their quantity.
As with voltage, an amplifier’s maximum current output may also be deliberately limited by internal protection circuitry in some designs.
[14] Non-acoustic | Output power
Output power is a more complex specification than it might initially seem, and interestingly, it’s not directly audible as a parameter.
What many don’t realize is that output power represents a thermal limit rather than an operational one. An amplifier rated at 100W can actually deliver 500W to speakers—it will just generate excessive heat when doing so. The power rating essentially indicates how much power an amplifier can deliver while maintaining thermal stability, though it may also be constrained by supply voltage limitations.
The common method of measuring power—taking maximum RMS output voltage and calculating current based on load impedance—is actually mathematically problematic. This “RMS power” concept is a misconception because RMS (root mean square) is a property of wave functions, while power itself is the integration of voltage with load impedance.
To standardize power ratings, the Audio Engineering Society (AES) established the “rated power” parameter. This represents the power an amplifier can deliver continuously for at least two hours without overheating, breaking, or engaging protection circuits. This specification is independent of load impedance because it fundamentally defines the amplifier’s heat dissipation capability. The AES standard also defines “maximum power” as double the rated power (+3dB).
Many assume higher output power means less signal saturation, but this isn’t necessarily true. Saturation or clipping results primarily from limitations in maximum output voltage and slew rate. Paradoxically, an amplifier capable of dissipating more power might actually reach its saturation point sooner than a lower-powered model if it has a lower supply voltage.
Like maximum current capability, an amplifier’s rated power directly relates to its output devices’ Safe Operating Area and the heat dissipation capacity of its cooling system.
[15] Non-acoustic | Power supply rejection ratio (PSRR)
The Power Supply Rejection Ratio (PSRR) measures an amplifier’s ability to maintain clean audio output despite imperfections in its power supply. This specification indicates how effectively the amplifier blocks noise, ripple, and other disturbances that originate from the power supply.
A higher PSRR value means the amplifier’s performance remains more consistent even when the power supply fluctuates or contains noise. This is particularly important in real-world conditions where power supplies are rarely perfect.
To measure PSRR, technicians ground the amplifier’s inputs and then deliberately introduce a variable frequency test voltage into one of the power supply lines. They then measure how much of this test signal appears at the amplifier’s output. The greater the attenuation of this unwanted signal, the better the amplifier’s PSRR performance.
PSRR typically decreases as frequency increases, making amplifiers more vulnerable to high-frequency power supply noise. Interestingly, amplifiers designed with substantial negative feedback generally demonstrate better PSRR because the feedback mechanism actively corrects for power supply fluctuations.
[16] Non-acoustic | Common mode rejection ratio (CMRR)
The Common Mode Rejection Ratio (CMRR) is a characteristic specific to amplifiers with differential inputs. It measures how effectively the amplifier ignores signals that appear identically on both input terminals while amplifying only the differences between them.
This specification indicates how well an amplifier can reject external interferences such as electromagnetic interference (EMI), as well as unwanted voltage variations and fluctuations that affect both inputs simultaneously. A higher CMRR value indicates superior performance in maintaining signal integrity despite environmental electrical noise.
[17] Non-acoustic | Load invariancy
Load invariancy describes an amplifier’s ability to maintain consistent performance regardless of what speaker is connected to it. An ideal amplifier would perform exactly the same whether connected to a purely resistive load, a reactive load, or a speaker whose impedance changes dramatically across the frequency spectrum.
In theory, an amplifier should be completely load-invariant. However, in reality, very few amplifiers actually achieve this ideal behavior.
You can observe how load-invariant an amplifier is by measuring the current difference between different impedance loads at the same output voltage. A theoretically perfect amplifier would exactly double its current output when the load impedance is halved. Real-world amplifiers never achieve this perfect relationship because their efficiency decreases and their input impedance changes under varying load conditions. High-quality amplifiers come close to this theoretical doubling, while lesser designs fall considerably short.
The damping factor specification also indicates how an amplifier handles varying load conditions. A low damping factor suggests weak control over the speaker and therefore greater performance variability when connected to different speaker loads.
[18] Non-acoustic | Compensation-Invariancy and amplifier stability
Compensation refers to techniques used to keep amplifiers stable at high frequencies. Without proper compensation, the internal components and stages would be prone to uncontrolled oscillations and would attempt to reproduce frequencies beyond their safe operating range.
Every active device in electronic circuits has a maximum frequency range it can handle before breaking down. Without adequate compensation, these components might attempt to deliver excessive voltage, current, or power (within their Safe Operating Area), potentially resulting in overheating or complete failure, especially in power output stages.
Designing effective compensation requires carefully positioned frequency “poles” and specific component choices. For example, high-quality NP0 (C0G) capacitors are often used because they maintain consistent capacitance regardless of voltage and temperature changes, and they introduce minimal additional distortion.
High-frequency stability depends on three critical factors: bandwidth with its associated phase characteristics, slew rate, and compensation. Interestingly, faster amplifiers with wider bandwidth aren’t automatically more prone to instability. An amplifier with restricted bandwidth and modest slew rate but poorly implemented compensation might actually be far less stable than a much faster, wider-bandwidth design that’s properly compensated.
A truly stable amplifier maintains its composure with any load connected to it. The most challenging test case is typically a capacitive load. Engineers test this by connecting a small capacitor (typically 1nF) directly to the amplifier output without any series resistance. This capacitor creates a response peak at the upper frequency limit of the amplifier. By examining the amplifier’s response to square waves, technicians can identify any ringing or oscillations that indicate stability problems.
This test helps predict how the amplifier will behave with no load connected or with distant speakers connected via long cables (which introduce significant capacitance).
An amplifier’s frequency response and phase characteristics can vary with temperature, internal component changes, and especially with different speaker loads. This is particularly noticeable in switching amplifiers (Class D, T, S, etc.), where frequency response often changes significantly as load impedance varies, since their output filters are designed for specific load impedances.
This variable performance relates directly to the amplifier’s load invariance. When connected to unusual loads, some amplifiers may develop oscillations due to unexpected phase shifts and frequency response changes.
[19] Non-acoustic | Thermal stability
Thermal stability refers to an amplifier’s ability to maintain consistent operating temperature and resist overheating that can lead to thermal drift—a change in performance as components heat up.
Interestingly, thermal drift can work in either direction. Some components, like lateral MOSFETs, exhibit negative thermal drift (performance changes in one direction as temperature increases). In contrast, BJT (Bipolar Junction Transistor) designs demonstrate positive thermal drift, with performance shifting in the opposite direction as they warm up.
A well-designed amplifier maintains relatively constant internal temperatures and, crucially, preserves its sonic characteristics even when temperature fluctuations do occur. If you encounter an amplifier that requires a “warm-up period” before it sounds its best, this generally indicates problematic design choices rather than sophisticated engineering.
Thermal stability directly relates to memory distortion: the more stable an amplifier’s temperature remains as signal levels change, the lower the resulting memory distortion. This stability ensures that transient performance remains consistent regardless of what was playing previously.
[20] Non-acoustic | Open loop gain and closed loop gain
Open loop gain is a fundamental characteristic of amplifiers with differential inputs. It refers to the amplification achieved when the inverting input is connected to ground. This specification reveals two important aspects of an amplifier: the amount of signal correction available at different frequencies and the maximum possible closed-loop gain the amplifier can provide.
By examining an amplifier’s open loop gain in relation to its phase response across different frequencies, engineers can determine its high-frequency stability—a critical factor for preventing oscillations.
In amplifiers without differential inputs, this characteristic is simply called “gain.” It quantifies how much the amplifier multiplies the input voltage to produce the output signal. For example, if an amplifier has 27dB of gain, it will multiply the input voltage by approximately 20 times.
In differential amplifiers, the actual gain (closed loop gain) is set by a voltage divider placed between the amplifier’s output and its second differential input. This closed loop gain must always be less than the open loop gain. There’s an important relationship here: higher gain settings typically reduce bandwidth, while lower gain settings can make the amplifier more susceptible to instability and can create frequency response peaks at high frequencies.
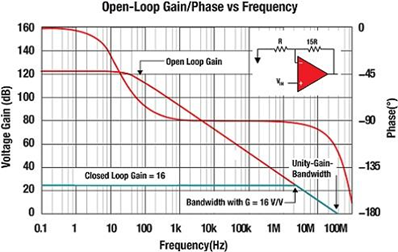
[21] Non-acoustic | Efficiency
Efficiency describes the relationship between the power an amplifier draws from the wall and the power it actually delivers to your speakers. This ratio is influenced by several key factors:
Supply voltage versus output voltage: Efficiency typically decreases as supply voltage increases. Additionally, when there’s a large difference between the supply voltage and the output voltage, efficiency suffers. This is why many high-efficiency designs use lower supply voltages or variable supply rails.
Operation class: The amplifier’s class (A, AB, D, etc.) fundamentally determines its maximum theoretical efficiency. This is why Class D amplifiers are preferred in applications where efficiency is paramount.
Quiescent current: Even when no music is playing, amplifiers draw some power—called quiescent current—which is continuously dissipated as heat. This creates a baseline inefficiency present at all times.
Load impedance: As speaker impedance decreases, amplifier efficiency typically decreases as well. This means an amplifier driving 4-ohm speakers will generally run less efficiently than when driving 8-ohm speakers.
Conclusions
As this overview demonstrates, properly evaluating an amplifier requires looking far beyond the commonly cited specifications of distortion, signal-to-noise ratio, and damping factor. The complete picture involves numerous additional parameters that each contribute significantly to real-world performance.
When all these specifications are understood together, they provide a comprehensive technical profile that can accurately predict how an amplifier will perform with specific speakers before any listening takes place. This scientific approach offers a much more reliable foundation for amplifier selection than relying on limited measurements or subjective impressions alone.